Abstract: We have developed a 1550 nm insulator-based lithium tantalate waveguide with a loss of 0.28 dB/cm and a ring resonator quality factor of 1.1 million. The application of χ(3) nonlinearity in nonlinear photonics has been studied. The advantages of lithium niobate on insulator (LNoI), which exhibits excellent χ(2) and χ(3) nonlinear properties along with strong optical confinement due to its "insulator-on" structure, have led to significant advances in waveguide technology for ultrafast modulators and integrated nonlinear photonics [1-3]. In addition to LN, lithium tantalate (LT) has also been investigated as a nonlinear photonic material. Compared to LN, LT has a higher optical damage threshold and a wider optical transparency window [4, 5], although its optical parameters, such as refractive index and nonlinear coefficients, are similar to those of LN [6, 7]. Thus, LToI stands out as another strong candidate material for high optical power nonlinear photonic applications. Moreover, LToI is becoming a primary material for surface acoustic wave (SAW) filter devices, applicable in high-speed mobile and wireless technologies. In this context, LToI wafers may become more common materials for photonic applications. However, to date, only a few photonic devices based on LToI have been reported, such as microdisk resonators [8] and electro-optic phase shifters [9]. In this paper, we present a low-loss LToI waveguide and its application in a ring resonator. Additionally, we provide the χ(3) nonlinear characteristics of the LToI waveguide.
Key Points:
• Offering 4-inch to 6-inch LToI wafers, thin-film lithium tantalate wafers, with top layer thicknesses ranging from 100 nm to 1500 nm, utilizing domestic technology and mature processes.
• SINOI: Ultra-low loss silicon nitride thin-film wafers.
• SICOI: High-purity semi-insulating silicon carbide thin-film substrates for silicon carbide photonic integrated circuits.
• LTOI: A strong competitor to lithium niobate, thin-film lithium tantalate wafers.
• LNOI: 8-inch LNOI supporting the mass production of larger-scale thin-film lithium niobate products.
Manufacturing on Insulator Waveguides: In this study, we utilized 4-inch LToI wafers. The top LT layer is a commercial 42° rotated Y-cut LT substrate for SAW devices, which is directly bonded to a Si substrate with a 3 µm thick thermal oxide layer, employing a smart cutting process. Figure 1(a) shows a top view of the LToI wafer, with the top LT layer thickness of 200 nm. We assessed the surface roughness of the top LT layer using atomic force microscopy (AFM).
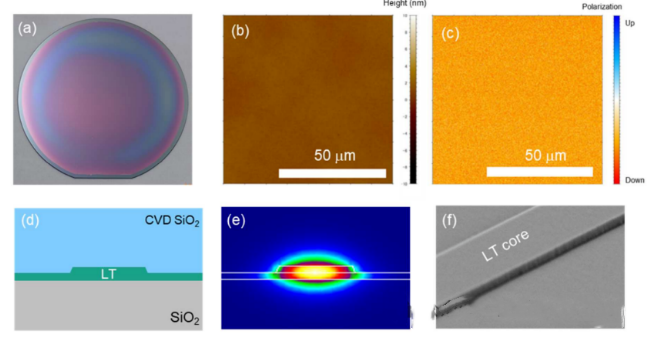
Figure 1. (a) Top view of the LToI wafer, (b) AFM image of the surface of the top LT layer, (c) PFM image of the surface of the top LT layer, (d) Schematic cross-section of the LToI waveguide, (e) Calculated fundamental TE mode profile, and (f) SEM image of the LToI waveguide core before SiO2 overlayer deposition. As shown in Figure 1 (b), the surface roughness is less than 1 nm, and no scratch lines were observed. Additionally, we examined the polarization state of the top LT layer using piezoelectric response force microscopy (PFM), as depicted in Figure 1 (c). We confirmed that uniform polarization was maintained even after the bonding process.
Using this LToI substrate, we fabricated the waveguide as follows. First, a metal mask layer was deposited for subsequent dry etching of the LT. Then, electron beam (EB) lithography was performed to define the waveguide core pattern on top of the metal mask layer. Next, we transferred the EB resist pattern to the metal mask layer via dry etching. Afterward, the LToI waveguide core was formed using electron cyclotron resonance (ECR) plasma etching. Finally, the metal mask layer was removed through a wet process, and a SiO2 overlayer was deposited using plasma-enhanced chemical vapor deposition. Figure 1 (d) shows the schematic cross-section of the LToI waveguide. The total core height, plate height, and core width are 200 nm, 100 nm, and 1000 nm, respectively. Note that the core width expands to 3 µm at the waveguide edge for optical fiber coupling.
Figure 1 (e) displays the calculated optical intensity distribution of the fundamental transverse electric (TE) mode at 1550 nm. Figure 1 (f) shows the scanning electron microscope (SEM) image of the LToI waveguide core before the deposition of the SiO2 overlayer.
Waveguide Characteristics: We first evaluated the linear loss characteristics by inputting TE-polarized light from a 1550 nm wavelength amplified spontaneous emission source into LToI waveguides of varying lengths. The propagation loss was obtained from the slope of the relationship between waveguide length and transmission at each wavelength. The measured propagation losses were 0.32, 0.28, and 0.26 dB/cm at 1530, 1550, and 1570 nm, respectively, as shown in Figure 2 (a). The fabricated LToI waveguides exhibited comparable low-loss performance to state-of-the-art LNoI waveguides [10].
Next, we assessed the χ(3) nonlinearity through the wavelength conversion generated by a four-wave mixing process. We input a continuous wave pump light at 1550.0 nm and a signal light at 1550.6 nm into a 12 mm long waveguide. As shown in Figure 2 (b), the phase-conjugate (idler) light wave signal intensity increased with increasing input power. The inset in Figure 2 (b) shows the typical output spectrum of the four-wave mixing. From the relationship between input power and conversion efficiency, we estimated the nonlinear parameter (γ) to be approximately 11 W^-1m.
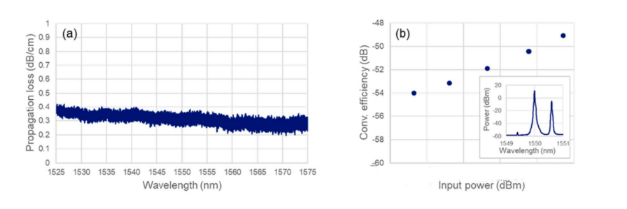
Figure 3. (a) Microscope image of the fabricated ring resonator. (b) Transmission spectra of the ring resonator with various gap parameters. (c) Measured and Lorentzian-fitted transmission spectrum of the ring resonator with a gap of 1000 nm.
Next, we fabricated an LToI ring resonator and evaluated its characteristics. Figure 3 (a) shows the optical microscope image of the fabricated ring resonator. The ring resonator features a "racetrack" configuration, consisting of a curved region with a radius of 100 µm and a straight region of 100 µm in length. The gap width between the ring and the bus waveguide core varies in increments of 200 nm, specifically at 800, 1000, and 1200 nm. Figure 3 (b) displays the transmission spectra for each gap, indicating that the extinction ratio changes with the gap size. From these spectra, we determined that the 1000 nm gap provides nearly critical coupling conditions, as it exhibits the highest extinction ratio of -26 dB.
Using the critically coupled resonator, we estimated the quality factor (Q factor) by fitting the linear transmission spectrum with a Lorentzian curve, obtaining an internal Q factor of 1.1 million, as shown in Figure 3 (c). To our knowledge, this is the first demonstration of a waveguide-coupled LToI ring resonator. Notably, the Q factor value we achieved is significantly higher than that of fiber-coupled LToI microdisk resonators [9].
Conclusion: We developed an LToI waveguide with a loss of 0.28 dB/cm at 1550 nm and a ring resonator Q factor of 1.1 million. The performance obtained is comparable to that of state-of-the-art low-loss LNoI waveguides. Additionally, we investigated the χ(3) nonlinearity of the manufactured LToI waveguide for on-chip nonlinear applications.
Post time: Nov-20-2024